Chapter 15. Population Genetics
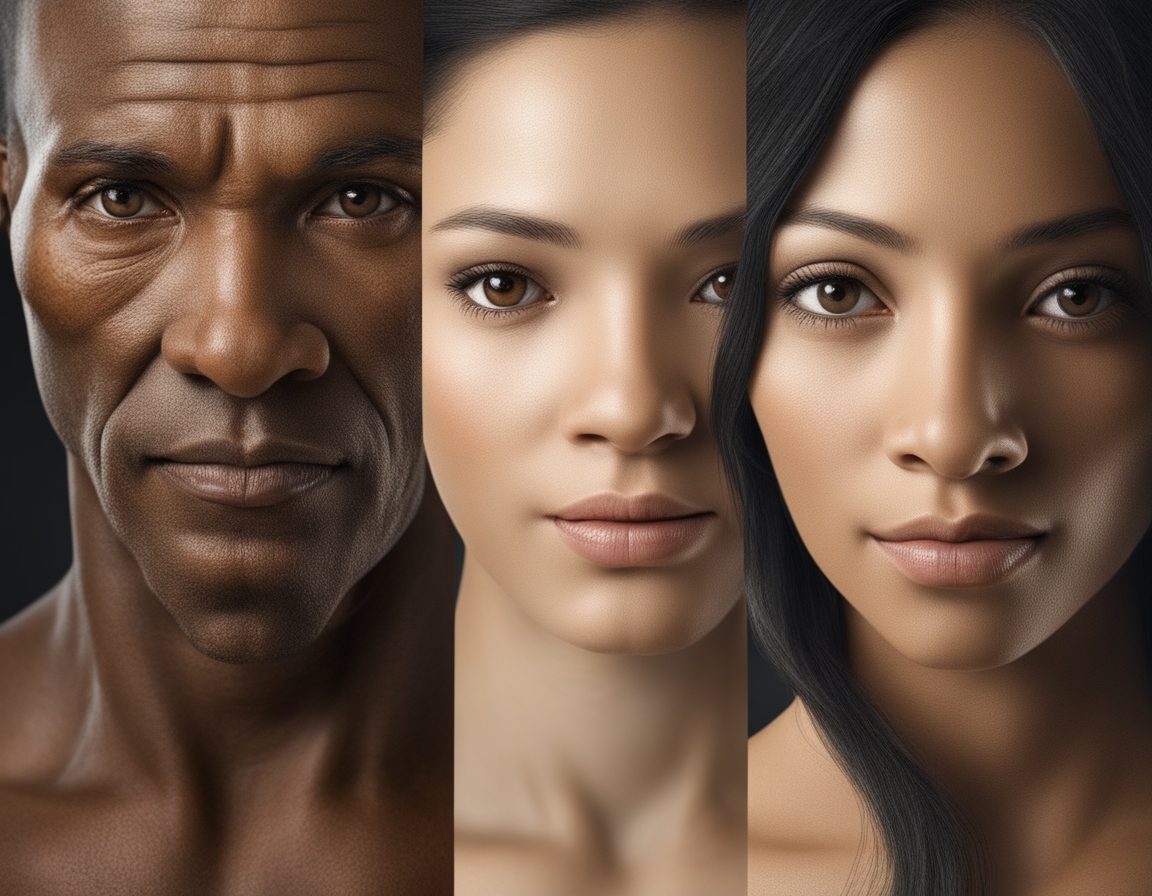
Population Genetics is the study of the distribution of genes in a population and of how the frequencies of genes and genotypes are maintained or changed.
A population is a localized group of individuals belonging to the same species. A species is a group of organisms capable of interbreeding and producing fertile offspring, and separated from other such groups with which interbreeding does not (normally) happen.
All humans on this planet belong to the Homo sapiens species. While there is variation in physical characteristics among humans, they exhibit a consistent set of morphological traits that distinguish them from other species. Human population genetics explores the genetic differences within and between populations to understand the genetic structure and evolutionary history of humans.
Human Genetic Variation
Although the human chromosomes and loci they contain are identical in all members of the human species, allele frequencies at many loci vary widely among population groups. Within each population there is extensive variation, greater on the average than the mean differences between groups. Most genetic variation in humans is found within populations. About 85-90% of genetic variation occurs within any given population. The remaining 10-15% of genetic variation exists between populations, reflecting differences in allele frequencies due to historical separation, adaptation to different environments, and genetic drift.
The typical difference between the genomes of two unrelated individuals is estimated at 20 million nucleotides (or 0.6% of the total of 3.2 billion nucleotides comprising the human genome). A single nucleotide polymorphism (SNP) is a difference in a single nucleotide between members of one species that occurs in at least 1% of the population. The 2,504 individuals characterized by the 1000 Genomes Project (https://www.internationalgenome.org/1000-genomes-summary) had 84.7 million SNPs among them.
SNPs represent the most common type of genetic variation among individuals. By analyzing SNPs, researchers can identify genetic differences that may be associated with specific traits or diseases. SNPs are used to study the genetic structure of populations, allowing researchers to infer population history, migration patterns, and ancestry. By comparing SNP frequencies across populations, scientists can trace lineage and evolutionary relationships. SNPs are crucial in genome-wide association studies (GWAS), which investigate the association between specific genetic variants and traits or diseases. Identifying SNPs linked to diseases can help understand the genetic basis of complex conditions and lead to the development of personalized medicine. In genetics, SNPs serve as markers for identifying regions of the genome associated with specific phenotypes. They are used in linkage analysis and quantitative trait locus (QTL) mapping to locate genes that contribute to particular traits. SNPs help in studying the evolutionary forces acting on populations, such as natural selection, genetic drift, and gene flow. By examining SNP variation, researchers can detect signals of adaptation and understand how populations have evolved over time. SNPs are used in forensic science for individual identification (Chapter 14) and in human evolutionary biology for studying human evolution and migration. They can provide information about the genetic makeup of ancient populations and their relationships to modern groups. SNPs play a role in pharmacogenomics, the study of how genetic variation influences individual responses to drugs. Identifying SNPs associated with drug metabolism can help tailor medical treatments to individual genetic profiles, improving drug efficacy and reducing adverse effects. Overall, SNPs are essential in population genetics for understanding genetic diversity, evolutionary processes, disease associations, and the genetic basis of traits, contributing significantly to various fields of biological research and medicine.
Although about 3% to 5% of human SNPs are located in the functional portion of the genome, it does not mean that these SNPs are functionally important, since many of them are in the third nucleotide of redundant codons (recall from Chapter 5 why does this have little effect on a polypeptide sequence).
Genetic variation underlies human adaptation to diverse environments. Genetic variation in humans is a result of mutation, recombination, gene flow, and genetic drift. The study of human population genetics reveals patterns of genetic diversity shaped by historical events, migration, and adaptation to different environments, providing insights into our evolutionary history and biological diversity.
Human populations are structured geographically, with genetic similarities correlating with geographical proximity. Populations that are geographically closer tend to be more genetically similar due to historical gene flow.
Hardy-Weinberg Equilibrium (HWE)
The Hardy-Weinberg Equilibrium (HWE) is a general rule for allelic behavior in a large population with random mating. It states that in an infinitely large, randomly mating population in which selection, migration, and mutation do not occur, the frequencies of alleles and genotypes do not change (that is, they are at equilibrium) from generation to generation. Since evolution is defined as a change in the frequency of an allele within a gene pool (Chapter 16), this means that a population in the HWE does not evolve.
In the simplest case of a single locus with two alleles: the dominant allele is denoted A and the recessive a and their frequencies are denoted by p and q; freq(A)=p; freq(a)=q; p+q=1. If the population is in equilibrium, then we will have freq(AA) = p2 for the AA homozygotes in the population, freq(aa) = q2 for the aa homozygotes, and freq(Aa) = 2pq for the heterozygotes.
A better, but equivalent, probabilistic description for the HWE is that the alleles for the next generation for any given individual are chosen randomly and independent of each other. Consider two alleles, A and a, with frequencies p and q, repectively, in the population. The different ways to form new genotypes can be derived using a Punnett square, where the fraction in each is equal to the product of the row and column probabilities.
These frequencies are called Hardy–Weinberg frequencies (or Hardy–Weinberg proportions). This is achieved in one generation, and only requires the assumption of random mating with an infinite population size.
It is important to understand that the infinite population size is a theoretical category and the Hardy-Weinberg principle is a theoretical model. That is, the HWE is impossible in nature. Genetic equilibrium is an ideal state that provides a baseline against which to measure change.
Factors affect the HWE include small population size, non-random mating (assortative mating, inbreeding), selection, mutation, and gene flow.
Changes in allele frequencies
Small population size can cause a random change in allele frequencies. This is due to a sampling effect, and is called genetic drift. Sampling effects are most important when population sizes are small or the allele is rare.
In small populations, genetic drift can cause alleles to become fixed (reach a frequency of 1) or lost (frequency of 0) much faster than in large populations. This leads to deviations from the expected genotype frequencies under HWE. Thus, the loss of genetic variation due to genetic drift can lead to increased homozygosity and fixation of alleles. For example, the Pingelapese people of Micronesia experienced a population bottleneck after a typhoon reduced their population to about 20 individuals. This led to a high frequency of a rare allele causing achromatopsia (complete color blindness caused by mutations in one of six autosomal recessive genes, which affects all aspects of daylight vision), illustrating the effect of genetic drift via bottleneck effect. A bottleneck effect occurs when a population’s size is drastically reduced for at least one generation due to an event such as a natural disaster, disease outbreak, or other events that affect the survival of individuals in a population. This reduction in population size results in a loss of genetic variation because only a small number of individuals contribute to the gene pool of subsequent generations.
Another type of genetic drift is caused by a founder effect. The founder effect occurs when a new population is established by a very small number of individuals from a larger population. This small group may carry only a fraction of the genetic diversity of the original population, leading to a new population with different allele frequencies compared to the original population. An example of the bottleneck effect in humans is the Afrikaner population of South Africa, which descended from a small number of Dutch settlers. The settlers carried a limited genetic diversity, resulting in higher frequencies of certain genetic disorders, like Huntington’s disease, within the Afrikaner population. Bottleneck and founder effects both reduce genetic diversity, and both effects can have lasting impacts on the genetic structure and health of human populations.
HWE and random mating in human populations
In human populations, mating can be random with respect to some traits, and nonrandom for others. Mating seems to be random for such characteristics as blood type groups (not observable). Mating may be nonrandom (assortative), such as showing preference for specific phenotypes for traits such as skin pigmentation and height (positive assortative mating). Mating can also be disassortative (negative assortative), displaying preference for dissimilar genotypes. Such type of mating is sometimes called a rare mate effect, or, more specifically, a rare male effect (negatively frequency-dependent male mating success). Negatively frequency-dependent male mating success demonstrates the complex interplay between natural selection and sexual selection. It underscores the idea that evolutionary fitness is not solely determined by inherent qualities of a trait but also by its relative frequency within the population. This dynamic fosters a balance where multiple traits can coexist and be maintained over time, contributing to the adaptive potential of the species.
Non-Random Mating: the Dutch’ story
(Adapted from By Richard Ingham, AFP, 2015)
The Netherlands is the land of giants: on average, its women stand almost 1.71 meters (5 feet 7 inches) tall, and its men 1.84 meters. But how the Dutch became the world’s tallest people has been somewhat of a mystery. After all, two centuries ago they were renowned for being among the shortest. What happened since then?
A popular explanation is nutrition — a calorie-stuffed diet rich in meat and dairy products. But that can’t be the whole story, experts say. Other European countries, too, have enjoyed similar prosperity and a rise in living standards, yet their citizens have not shot skywards as much.
The average male height in the Netherlands has gained 20 cm (eight inches) in the last 150 years, according to military records. By comparison, the height of the average American man has risen a mere six cm over the same period.
Researchers led by Gert Stulp, a specialist in population health at the London School of Hygiene and Tropical Medicine, combed a Dutch database for clues. Called LifeLines, the record contains exhaustive detail about the lives and health of more than 94,500 people who lived in the northern the Netherlands from 1935 to 1967. In this three-decade snapshot, the people who had the most children were tall men, and women of average height, the team found. For example, the most fertile men were 7 cm above the average height. Statistically, they had 0.24 more children on average than the least fertile men, who were about 14 cm below the average height.
Compared to counterparts in other countries where they often tended to have fewer children, taller women also reproduced more in the Netherlands. Many postponed having children until after their studies, but once they forged a successful relationship, often had a large family. The study did not involve genetic testing, but concluded from the observations that natural selection must have played a part: with time, more and more Dutch started sporting tall genes.
“Natural selection in addition to good environmental conditions may help explain why the Dutch are so tall,” said the study published in the Royal Society journal Proceedings B (Stulp et al., 2015).
“Height is very heritable — taller parents tend to have somewhat taller children than shorter parents,” Stulp told AFP by email. “Because taller individuals would have more offspring in the next generation who would be taller, the average height in that generation would a bit taller on average than the preceding generation, if all else is equal.”
There seems to be a cultural preference as well. Stulp pointed to figures showing that, in the United States, shorter women and men of average height have the most reproductive success. “There is much variation in what men and women want,” he said.
“When it comes to choosing a mate, height tends to have (only) a small effect, which is not very surprising given the many other, more important, traits people value in their mate.”
Inbreeding and the frequency of rare traits
Inbreeding describes matings between individuals who are related by descent from a common ancestor. Both assortative mating and inbreeding can bring about an increase in the frequency of both homozygous genotypes and a decrease in the corresponding heterozygous form. Such an increase of homozygosity can lead to inbreeding depression, where deleterious recessive alleles become more common. This shifts genotype frequencies away from HWE expectations.
Selection
Natural selection acts on individuals, affecting their survival and reproductive success relative to others in the population. Selection, in general, causes allele frequencies to change, often quite rapidly, due to their effect on organismal fitness. While directional selection (a mode of natural selection in which a single phenotype is favored, causing the allele frequency to continuously shift in one direction) eventually leads to the loss of all alleles except the favored one, some forms of selection, such as balancing selection, lead to equilibrium without loss of alleles.
Positive natural selection, the mechanism by which advantageous traits become more common in a population, drives adaptive evolution. For a trait to be positively selected, it must meet two criteria. First, the trait must confer a benefit, increasing the organism’s likelihood of survival and reproduction. Second, the trait must be heritable, allowing it to be transmitted to the organism’s offspring. Beneficial traits can be diverse, ranging from protective coloration and the ability to exploit a new food source, to morphological changes that enhance survival in a specific environment. When a beneficial trait leads to a higher number of offspring who inherit it, that trait becomes more prevalent in the population over time compared to a randomly arising trait.
At the molecular level, positive selection manifests when a specific DNA variant becomes more frequent due to its advantageous impact on the organisms carrying it. In the early (pre-DNA) days of the development of evolutionary biology, Charles Darwin and Alfred Russel Wallace (1858) famously suggested that positive selection could account for the numerous adaptations aligning organisms with their environments and lifestyles, a concept that remains the cornerstone of evolutionary adaptation today.
However, positive selection is not the sole component of evolution. In humans, most mutations are considered selectively neutral, neither benefiting nor harming their carriers. The frequencies of these neutral genetic variants, or alleles, often increase by chance through genetic drift, which is thought to be the predominant mechanism in human evolution (Kimura, 1968). Additionally, when selection occurs, it is typically in the form of negative or purifying selection. This process eliminates new harmful mutations as they appear, rather than promoting the spread of advantageous traits. At the same time, there are many examples of positive selection in human populations, involving such traits as lactose tolerance, high altitude adaptation, and disease resistance.
Mutation
Mutation is the primary mechanism by which new alleles can enter a population. At the same time, mutation will have a very subtle effect on allele frequencies. Mutation rates are of the order 10−4 to 10−8, and the change in allele frequency will be, at most, the same order. Recurrent muta- tion will maintain alleles in the population, even if there is strong selection against them.
Migration and gene flow
Migration genetically links two or more populations together. In general, allele frequencies will become more homogeneous among the populations due to migration. Some models for migration inherently include nonrandom mating (Wahlund effect, for example). For those models, the Hardy–Weinberg proportions will normally not be valid.
Gene flow is defined as gradual diffusion of genes from one population to another by migration and mating. Gene flow introduces new alleles into a population, increasing genetic diversity. This can enhance the overall genetic variation within a population, providing a greater reservoir of genetic traits that may be beneficial in adapting to changing environments. A classic example of gene flow is the transfer of genetic loci between ethnic groups. Increased interbreeding between different ethnic groups often results in higher levels of heterozygosity, which can have beneficial effects on population health, such as reducing the likelihood of recessive genetic disorders. Gene flow can introduce alleles that confer advantages in particular environments, aiding in the adaptation of populations to local conditions. For instance, the introduction of alleles related to lactose tolerance or high-altitude adaptation from one population to another can provide immediate adaptive benefits. On the other hand, small island populations, like those in the Pacific, which were formed via founder effect, can experience substantial genetic changes with the arrival of new individuals from other islands or mainland areas, which may lead to a reduction to specific adaptations of the islanders to their island-specific environment.
Increased genetic admixture complicates the study of population genetics, as it becomes harder to attribute genetic traits to specific ancestral groups. This complexity must be accounted for in genetic research and ancestry testing. Furthermore, the blending of genetic material is often accompanied by cultural exchanges and shifts in social structures. It can influence social dynamics, cultural identities, and perceptions of ethnicity. As genetic research highlights the extensive intermixing of human populations, it challenges simplistic notions of race and ethnicity, promoting a more nuanced understanding of human genetic diversity.
Ethnic Groups and Human Races
From a biological prospective, race is a synonym to population and is defined as a group of organisms that can interbreed and differs from other groups in the frequency of certain hereditary traits.
The nineteenth-century idea that there are only three human races — Caucasoid, Mongoloid, and Negroid — emerged from European folk concepts of the Middle Ages about the significance of physical differences like skin pigmentation, head shape, or type of hair. All people were thought to belong to one of these races and most authorities believed that these physical differences also implied differences in intelligence, abilities, and general merit as human beings. Some believed that such “racial” differences justified social inferiority, colonial control, and even slavery.
Louis Agassiz, a 19th-century Harvard professor of zoology and geology, is the father of scientific racism. The foundation of his racial distinction was the concept of polygenism, which assumes that different races have separate geographic and biological origins, essentially designating different races as different species. This pattern of thinking led some US politicians of the early 20th century to argue that multiracial marriages would be childless, due to biological incompatibility among human races, and those children that do get born would be a danger to society. These ideas led the Commonwealth of Virginia to outlaw marriages between “whites” and any other race, where the latter was defined as having even one drop of “non-white blood” (Virginia’s 1924 Racial Integrity Act).
The concept of race in humans is socio-political and biologically non-applicable. Genetic research has shown that human populations cannot be divided into clearly defined, biologically distinct groups. In the last 200+ years, genetic flow among different formerly isolated human populations increased and frequencies of many genetic traits became indistinguishable between individuals from different populations. Phenotypic differences began to disappear as well.
All modern humans are related to a common ancestral group that lived in Africa 100,000 years ago. We know about the latter through the research on mtDNA. Most humans who live outside of Africa today also have a small amount of genetic ancestry from our extinct biological cousins, Neanderthals and Denisovans, with whom we share common ancestry. Human evolutionary genetics is the subject of Chapter 16.
Key Takeaways
- In a large population with no external sources influencing the allelic and genotypic frequency, these frequencies remain constant from generation to generation.
- Allelic and phenotypic frequencies are affected the population size, the mating strategy, as well as on the incidence of positive or negative selection and the influx of new mutations.